Functionalization, preparation and use of cell-laden gelatin methacryloyl–based hydrogels as modular tissue culture platforms
Progress in advancing a system-level understanding of the complexity of human tissue development and regeneration is hampered by a lack of biological model systems that recapitulate key aspects of these processes in a physiological context. Hence, growing demand by cell biologists for organ-specific extracellular mimics has led to the development of a plethora of 3D cell culture assays based on natural and synthetic matrices. We developed a physiological microenvironment of semisynthetic origin, called gelatin methacryloyl (GelMA)-based hydrogels, which combine the biocompatibility of natural matrices with the reproducibility, stability and modularity of synthetic biomaterials. We describe here a step-by-step protocol for the preparation of the GelMA polymer, which takes 1–2 weeks to complete, and which can be used to prepare hydrogel-based 3D cell culture models for cancer and stem cell research, as well as for tissue engineering applications. We also describe quality control and validation procedures, including how to assess the degree of GelMA functionalization and mechanical properties, to ensure reproducibility in experimental and animal studies.
This is a preview of subscription content, access via your institution
Access options
Subscribe to this journal
Receive 12 print issues and online access
265,23 € per year
only 22,10 € per issue
Buy this article
- Purchase on SpringerLink
- Instant access to full article PDF
Prices may be subject to local taxes which are calculated during checkout
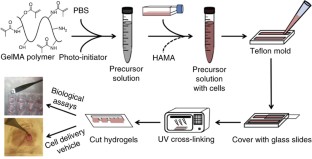
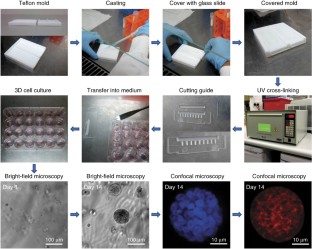
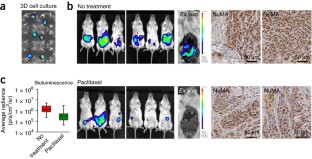
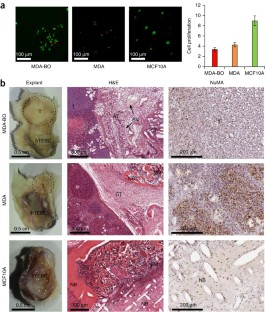
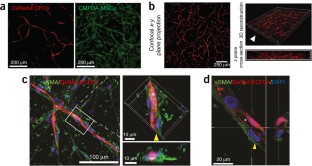
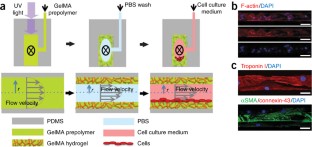
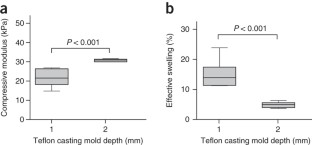
Similar content being viewed by others
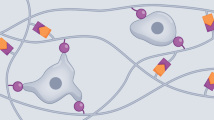
Chemical strategies to engineer hydrogels for cell culture
Article 30 August 2022
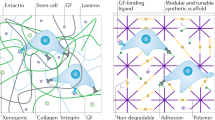
Synthetic alternatives to Matrigel
Article 27 May 2020
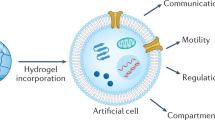
Hydrogels as functional components in artificial cell systems
Article 27 July 2022
References
- Wolf, K. et al. Collagen-based cell migration models in vitro and in vivo. Semin. Cell Dev. Biol.20, 931–941 (2009). ArticleCASGoogle Scholar
- Schwarzbauer, J. Basement membranes: putting up the barriers. Cur. Biol.9, R242–R244 (1999). ArticleCASGoogle Scholar
- Hynes, R.O. The extracellular matrix: not just pretty fibrils. Science326, 1216–1219 (2009). ArticleCASGoogle Scholar
- Frantz, C., Stewart, K.M. & Weaver, V.M. The extracellular matrix at a glance. J. Cell Sci.123, 4195–4200 (2010). ArticleCASGoogle Scholar
- Lu, P., Takai, K., Weaver, V.M. & Werb, Z. Extracellular matrix degradation and remodeling in development and disease. Cold Spring Harb. Perspect. Biol.3, a005058 (2011). ArticleGoogle Scholar
- Kutys, M.L. & Yamada, K.M. An extracellular-matrix-specific GEF-GAP interaction regulates Rho GTPase crosstalk for 3D collagen migration. Nat. Cell Biol.16, 909–917 (2014). ArticleCASGoogle Scholar
- Bissell, M.J. & Radisky, D. Putting tumours in context. Nat. Rev. Cancer1, 46–54 (2001). ArticleCASGoogle Scholar
- Wirtz, D., Konstantopoulos, K. & Searson, P.C. The physics of cancer: the role of physical interactions and mechanical forces in metastasis. Nat. Rev. Cancer11, 512–522 (2011). ArticleCASGoogle Scholar
- Pickup, M.W., Mouw, J.K. & Weaver, V.M. The extracellular matrix modulates the hallmarks of cancer. EMBO Rep.15, 1243–1253 (2014). ArticleCASGoogle Scholar
- Levett, P.A. et al. A biomimetic extracellular matrix for cartilage tissue engineering centered on photocurable gelatin, hyaluronic acid and chondroitin sulfate. Acta Biomater.10, 214–223 (2014). ArticleCASGoogle Scholar
- Engler, A.J., Sen, S., Sweeney, H.L. & Discher, D.E. Matrix elasticity directs stem cell lineage specification. Cell126, 677–689 (2006). ArticleCASGoogle Scholar
- Huebsch, N. et al. Harnessing traction-mediated manipulation of the cell/matrix interface to control stem-cell fate. Nat. Mater.9, 518–526 (2010). ArticleCASGoogle Scholar
- Loessner, D. et al. Bioengineered 3D platform to explore cell-ECM interactions and drug resistance of epithelial ovarian cancer cells. Biomaterials31, 8494–8506 (2010). ArticleCASGoogle Scholar
- Nichol, J.W. et al. Cell-laden microengineered gelatin methacrylate hydrogels. Biomaterials31, 5536–5544 (2010). ArticleCASGoogle Scholar
- Chaudhuri, O. et al. Extracellular matrix stiffness and composition jointly regulate the induction of malignant phenotypes in mammary epithelium. Nat. Mater.13, 970–978 (2014). ArticleCASGoogle Scholar
- Hutmacher, D.W. Biomaterials offer cancer research the third dimension. Nat. Mater.9, 90–93 (2010). ArticleCASGoogle Scholar
- Hutmacher, D.W. et al. Can tissue engineering concepts advance tumor biology research? Trends Biotechnol.28, 125–133 (2010). ArticleCASGoogle Scholar
- Loessner, D., Holzapfel, B.M. & Clements, J.A. Engineered microenvironments provide new insights into ovarian and prostate cancer progression and drug responses. Adv. Drug Deliv. Rev.79–80, 193–213 (2014). ArticleGoogle Scholar
- Malda, J. et al. 25th anniversary article: engineering hydrogels for biofabrication. Adv. Mater.25, 5011–5028 (2013). ArticleCASGoogle Scholar
- Annabi, N. et al. 25th anniversary article: rational design and applications of hydrogels in regenerative medicine. Adv. Mater.26, 85–123 (2014). ArticleCASGoogle Scholar
- Bichsel, C.A. et al. Diagnostic microchip to assay 3D colony-growth potential of captured circulating tumor cells. Lab Chip12, 2313–2316 (2012). ArticleCASGoogle Scholar
- Bray, L.J. et al. Multi-parametric hydrogels support 3D in vitro bioengineered microenvironment models of tumour angiogenesis. Biomaterials53, 609–620 (2015). ArticleCASGoogle Scholar
- Loessner, D. et al. A bioengineered 3D ovarian cancer model for the assessment of peptidase-mediated enhancement of spheroid growth and intraperitoneal spread. Biomaterials34, 7389–7400 (2013). ArticleCASGoogle Scholar
- Szot, C.S., Buchanan, C.F., Freeman, J.W. & Rylander, M.N. 3D in vitro bioengineered tumors based on collagen I hydrogels. Biomaterials32, 7905–7912 (2011). ArticleCASGoogle Scholar
- Kleinman, H.K. & Martin, G.R. Matrigel: basement membrane matrix with biological activity. Semin. Cancer Biol.15, 378–386 (2005). ArticleCASGoogle Scholar
- Hutmacher, D.W. & Cukierman, E. Engineering of tumor microenvironments. Adv. Drug Deliv. Rev.79–80, 1–2 (2014). ArticleGoogle Scholar
- Ehrbar, M. et al. Biomolecular hydrogels formed and degraded via site-specific enzymatic reactions. Biomacromolecules8, 3000–3007 (2007). ArticleCASGoogle Scholar
- Serebriiskii, I., Castello-Cros, R., Lamb, A., Golemis, E.A. & Cukierman, E. Fibroblast-derived 3D matrix differentially regulates the growth and drug-responsiveness of human cancer cells. Matrix Biol.27, 573–585 (2008). ArticleCASGoogle Scholar
- Kenny, H.A., Krausz, T., Yamada, S.D. & Lengyel, E. Use of a novel 3D culture model to elucidate the role of mesothelial cells, fibroblasts and extra-cellular matrices on adhesion and invasion of ovarian cancer cells to the omentum. Int. J. Cancer121, 1463–1472 (2007). ArticleCASGoogle Scholar
- Kenny, H.A. et al. Quantitative high throughput screening using a primary human three-dimensional organotypic culture predicts in vivo efficacy. Nat. Commun.6, 6220 (2015). ArticleCASGoogle Scholar
- Chwalek, K., Tang-Schomer, M.D., Omenetto, F.G. & Kaplan, D.L. In vitro bioengineered model of cortical brain tissue. Nat. Protoc.10, 1362–1373 (2015). ArticleCASGoogle Scholar
- Jenkins, J., Dmitriev, R.I., Morten, K., McDermott, K.W. & Papkovsky, D.B. Oxygen-sensing scaffolds for 3-dimensional cell and tissue culture. Acta Biomater.16, 126–135 (2015). ArticleCASGoogle Scholar
- Sieh, S. et al. Paracrine interactions between LNCaP prostate cancer cells and bioengineered bone in 3D in vitro culture reflect molecular changes during bone metastasis. Bone63, 121–131 (2014). ArticleCASGoogle Scholar
- Gomez-Guillen, M.C., Gimenez, B., Lopez-Caballero, M.E. & Montero, M.P. Functional and bioactive properties of collagen and gelatin from alternative sources: a review. Food Hydrocolloid.25, 1813–1827 (2011). ArticleCASGoogle Scholar
- Van Den Bulcke, A.I. et al. Structural and rheological properties of methacrylamide modified gelatin hydrogels. Biomacromolecules1, 31–38 (2000). ArticleCASGoogle Scholar
- Sutter, M., Siepmann, J., Hennink, W.E. & Jiskoot, W. Recombinant gelatin hydrogels for the sustained release of proteins. J. Control. Release119, 301–312 (2007). ArticleCASGoogle Scholar
- Kaemmerer, E. et al. Gelatine methacrylamide-based hydrogels: an alternative three-dimensional cancer cell culture system. Acta Bomater.10, 2551–2562 (2014). ArticleCASGoogle Scholar
- Yue, K. et al. Synthesis, properties, and biomedical applications of gelatin methacryloyl (GelMA) hydrogels. Biomaterials73, 254–271 (2015). ArticleCASGoogle Scholar
- Schuurman, W. et al. Gelatin-methacrylamide hydrogels as potential biomaterials for fabrication of tissue-engineered cartilage constructs. Macromol. Biosci.13, 551–561 (2013). ArticleCASGoogle Scholar
- Chen, Y.C. et al. Functional human vascular network generated in photocrosslinkable gelatin methacrylate hydrogels. Adv. Funct. Mater.22, 2027–2039 (2012). ArticleCASGoogle Scholar
- Bertassoni, L.E. et al. Hydrogel bioprinted microchannel networks for vascularization of tissue engineering constructs. Lab Chip.14, 2202–2211 (2014). ArticleCASGoogle Scholar
- Paul, A. et al. Injectable graphene oxide/hydrogel-based angiogenic gene delivery system for vasculogenesis and cardiac repair. ACS Nano8, 8050–8062 (2014). ArticleCASGoogle Scholar
- Thibaudeau, L. et al. Mimicking breast cancer-induced bone metastasis in vivo: current transplantation models and advanced humanized strategies. Cancer Metastasis Rev.33, 721–735 (2014). ArticleCASGoogle Scholar
- Annabi, N. et al. Hydrogel-coated microfluidic channels for cardiomyocyte culture. Lab Chip13, 3569–3577 (2013). ArticleCASGoogle Scholar
- Gong, J.P. Friction and lubrication of hydrogels: its richness and complexity. Soft Matter2, 544–552 (2006). ArticleCASGoogle Scholar
- O'Brien, A.K., Cramer, N.B. & Bowman, C.N. Oxygen inhibition in thiol–acrylate photopolymerizations. J. Polym. Sci. A Polym. Chem.44, 2007–2014 (2006). ArticleCASGoogle Scholar
- Bartnikowski, M., Bartnikowski, N.J., Woodruff, M.A., Schrobback, K. & Klein, T.J. Protective effects of reactive functional groups on chondrocytes in photocrosslinkable hydrogel systems. Acta Biomater.27, 66–76 (2015). ArticleCASGoogle Scholar
- Levett, P.A., Hutmacher, D.W., Malda, J. & Klein, T.J. Hyaluronic acid enhances the mechanical properties of tissue-engineered cartilage constructs. PLoS ONE9, e113216 (2014). ArticleGoogle Scholar
- Hollander, A.P. et al. Increased damage to type II collagen in osteoarthritic articular cartilage detected by a new immunoassay. J. Clin. Invest.93, 1722–1732 (1994). ArticleCASGoogle Scholar
Acknowledgements
The work presented by the authors was supported by the Australian Research Council (Future Fellowship awarded to T.J.K. and D.W.H.; Discovery Project grants awarded to D.L., T.J.K. and D.W.H.), the National Health and Medical Research Council of Australia (D.W.H.), Cancer Council Queensland (D.L.), the European Union (Marie Curie Fellowship PIOF-GA-2010-272286 to F.P.W.M.), the US National Science Foundation (EFRI-1240443 to A.K.), IMMODGEL (602694 to A.K.) and the US National Institutes of Health (EB012597, AR057837, DE021468, HL099073, AI105024 and AR063745 to A.K.). We also acknowledge the National Breast Cancer Foundation (IN-15-047 to D.W.H.) Foundation (IN-15-047 to D.W.H.) and an Australia-Harvard Fellowship (to A.K. and D.W.H.) from Harvard Club of Australia Foundation. Australia-Harvard Fellowship (to A.K. and D.W.H.) Harvard Club of Australia Foundation.
Author information
- Daniela Loessner and Christoph Meinert: These authors contributed equally to this work.
Authors and Affiliations
- Queensland University of Technology (QUT), Brisbane, Queensland, Australia Daniela Loessner, Christoph Meinert, Elke Kaemmerer, Laure C Martine, Peter A Levett, Travis J Klein, Ferry P W Melchels & Dietmar W Hutmacher
- Division of Biomedical Engineering, Department of Medicine, Biomaterials Innovation Research Center, Brigham and Women's Hospital, Harvard Medical School, Boston, Massachusetts, USA Kan Yue & Ali Khademhosseini
- Division of Health Sciences and Technology, Harvard-Massachusetts Institute of Technology, Massachusetts Institute of Technology, Cambridge, Massachusetts, USA Kan Yue & Ali Khademhosseini
- Department of Orthopaedics, University Medical Center Utrecht, Utrecht, the Netherlands Ferry P W Melchels
- Institute of Biological Chemistry, Biophysics and Bioengineering, School of Engineering and Physical Sciences, Heriot-Watt University, Edinburgh, UK Ferry P W Melchels
- Wyss Institute for Biologically Inspired Engineering, Harvard University, Boston, Massachusetts, USA Ali Khademhosseini
- Department of Bioindustrial Technologies, College of Animal Bioscience and Technology, Konkuk University, Hwayang-dong, Gwangjin-gu, Seoul, Republic of Korea Ali Khademhosseini
- Department of Physics, King Abdulaziz University, Jeddah, Saudi Arabia Ali Khademhosseini
- Australian Prostate Cancer Research Centre-Queensland, Translational Research Institute, Queensland University of Technology, Brisbane, Queensland, Australia Dietmar W Hutmacher
- George W. Woodruff School of Mechanical Engineering, Georgia Institute of Technology, Atlanta, Georgia, USA Dietmar W Hutmacher
- Institute for Advanced Study, Technische Universität München, Munich, Germany Dietmar W Hutmacher
- Daniela Loessner